Authored by Evan Peikon*,
Abstract
The relationship between power output and exercise tolerance is well described by a hyperbolic equation, establishing the critical power (CP) and the curvature constant (W’). Critical power represents the greatest metabolic rate that results in ‘wholly-oxidative’ energy provision. Wholly-oxidative considers the active organism in toto and means that energy supply through substrate-level phosphorylation reaches a steadystate, indicated by a lack of progressive intramuscular phosphocreatine breakdown. W’ was initially described as an anaerobic work capacity but has subsequently been shown to be associated with the depletion of intramuscular energy stores as well as to be sensitive to alterations in oxygen delivery. Taken together, these two findings are consistent with the hypothesis that muscle oxygen saturation (SmO2), as measured by near-infrared spectroscopy (NIRS), is a viable means of estimating the power-duration relationship, and subsequently, time to exhaustion at a fixed pace.
Introduction
For something so familiar that everyone has experienced it, fatigue is paradoxically challenging to define. This is partly due to the limits of language and the fact that different fields of science define fatigue differently. But, it’s also due to the complexity of all of the underlying processes that lead to fatigue. For much of the time that ‘fatigue science’ has been a field, the ‘catastrophic’ model of fatigue was used to describe what occurs when an athlete reaches the absolute limit of their physical performance. Proponents of this model assert that the body either runs out of key nutrients or is ‘poisoned’ due to metabolite accumulation in the working skeletal muscles. However, as early as the dawn of the twentieth century, some individuals challenged these assumptions, one such example being Angelo Mosso, who stated, “At first sight [fatigue] might appear an imperfection of our body, is on the contrary one of its most marvelous perfections. The fatigue increases more rapidly than the amount of work done saves us from the injury which lesser sensibility would involve for the organism” [1]. In this view, fatigue is an immensely complex derivative of a number of functions, behaviors, and psychological processes. As a result, exercise limitations involve a wide range of systems working together in harmony to maintain homeostasis [2].
While these descriptive views of fatigue and exercise limitations can be useful, they do not improve practitioners’ ability to predict or manage fatigue in athletes. As a result, the link between fatigue and performance has always been elusive. However, in recent years compelling evidence has indicated that the relationship between fatigue and performance is enshrined in the concept of critical power (CP). At its essence, this concept describes the tolerable duration of severe-intensity exercise and a finite amount of work that can be done above critical power, which is represented by the curvature constant, W’. W’ has traditionally been described as an anaerobic work capacity [3]. However, there is evidence to suggest that W’ is sensitive to oxygen delivery [4]. By looking at critical power and W’ through the lens of oxygen delivery and utilization, two of the major determinants of exercise capacity, we can gain a new perspective that better allows us to describe and predict fatigue in athletes [5].
This paper reviews the evidence that critical power is influenced by the balance of oxygen delivery and utilization in working muscles and that the curvature constant, W’, is related to regional oxygen saturation in said muscles. As a result, nearinfrared spectroscopy may be a viable means of estimating the power-duration relationship and time to exhaustion at a fixed pace in live time.
Critical Power: An Important Fatigue Threshold
What is critical power and how is it calculated?
Critical power is mathematically defined as the powerasymptote of the hyperbolic relationship between power output and time to exhaustion [6]. In essence, critical power describes the duration that an individual can sustain a fixed power output in the severe exercise intensity domain, and physiologically critical power represents the boundary between steady-state and nonsteady- state exercise [6]. As a result, critical power may provide a more meaningful fitness index over more well-known performance metrics such as VO2max or lactate balance point. The hyperbolic equation which describes the relationship between power output and exercise tolerance within the severe exercise intensity domain is as follows: t=W’/(P-CP), where (t) stands for time, (P) and (CP) stands for power and critical power respectively, and (W’) represents the curvature constant [3,7,8]. This equation creates a two-parameter model where critical power represents the asymptote for power, and the W’ represents a finite amount of work that can be done above critical power [9]. Taken together, these two parameters can be used to predict the tolerable duration of exercise above critical power. The hyperbolic equation describing the power-duration relationship is rigorous and conserved across different forms of exercise, individual muscles, and modalities, thus establishing it as an important fatigue threshold for athletes in a range of sporting disciplines [10] (Figure 1).

There are currently two validated methods for determining critical power and the fixed amount of work that can be done above critical power, termed W’. Traditionally critical power and W’ were calculated after having an individual perform three to seven all-out work bouts where they hold a fixed power-output until failure [11]. These test results are then plotted on a chart where the x, y variables represent time to failure and power for each trial. Critical power is then determined as the slope of the work-time relationship, whereas W’ is determined from the y-intercept [7]. More recently, though, investigators have introduced a 3-minute all-out exercise test, known as the 3MT, that has enabled the determination of critical power and W’ from a single exercise bout [12,13]. The idea behind the 3-minute all-out test is that when a subject exerts themselves fully and expends W’ wholly, their power output equals their critical power [10]. This idea can be summarized and expressed with the following equation: P=(W’/t lim)+CP.
Using these methods, critical power was originally defined as the external power output that could be sustained ‘indefinitely’ [14]. However, it should be understood that this definition is mainly theoretical as no bout of exercise can be sustained for an indefinite period regardless of the intensity. As a result, we can better understand critical power as the highest power output that can be sustained for a very long period of time without fatigue. In contrast to the historical definition, critical power is now considered to represent the greatest metabolic rate that results in ‘wholly oxidative’ energy provision, where wholly-oxidative considers the active organism as a whole. This means that energy supply through substrate-level phosphorylation reaches a steadystate and that there is no progressive accumulation of blood lactate or progressive breakdown of intramuscular phosphocreatine [14]. Given that HbO2 saturation, as measured with near-infrared spectroscopy, approximates phosphocreatine kinetics measured with magnetic resonance spectroscopy, we can conclude that a relationship between critical power and HbO2 saturation exists [15]. As a result, the balance of oxygen supply and demand, which are two of the major determinants of exercise performance, can be used as a means of understanding critical power and W’. The balance of oxygen supply and demand can be understood through near-infrared spectroscopy, also known as NIRS.
What is NIRS and what does it measure?
NIRS stands for near-infrared spectroscopy. NIRS is a technology that allows one to measure in vivo oxidative metabolism in human skeletal muscle. A NIRS device consists of a light source emitting two or more wavelengths in the near-infrared range of 650- 1000nm and a detector placed at a known distance from the light source. Since near-infrared light can penetrate biological tissues with less scattering and absorption than visible light, it offers many advantages for imaging and quantitative measurements. These quantitative measurements depend on the physics principle of reflectance, which is outlined in the Beer-Lambert law. This law states that certain materials attenuate the transmission of light at specific wavelengths, and when this equation is adapted to match the properties of human muscle, it allows one to measure changes in oxygenated and deoxygenated hemoglobin concentrations within a given muscle. This is made possible because the chromophores hemoglobin and myoglobin are oxygen carriers in the blood and skeletal myocytes, respectively, and their absorbance of nearinfrared light depends on whether they are in an oxygenated or deoxygenated state [5]. As a result, NIRS measurements can be used to reflect the balance of oxygen delivery to the working muscles and oxygen consumption in the capillary beds [16]. Additionally, tissue oximeters can measure regional oxygenated hemoglobin and myoglobin saturation, which represents tissue reserve capacity following tissue oxygen extraction [17]. This makes NIRS a very useful tool for assessing two of the major determinants of exercise capacity: oxygen delivery and oxygen utilization, respectively [5].
What is the relationship between critical power and oxygen delivery?
As stated previously, critical power is the maximal power output at which a metabolic steady state characterized by stable intracellular levels of adenosine triphosphate, phosphocreatine, hydrogen ions, inorganic phosphate, and blood lactate are reached [18-20]. When exercising above critical power, one begins to deplete W’, which is characterized by a finite amount of work that can be done above critical power. W’ was initially described as an anaerobic work capacity but has subsequently been shown to be associated with the depletion of intramuscular energy stores and is sensitive to alterations in oxygen delivery [3,4]. When exercising at a fixed supra-critical power output, VO2, intracellular inorganic phosphates, and blood lactate will progressively increase until exhaustion, at which point VO2 reaches a maximum value, termed VO2max [21].
VO2max refers to the maximum rate of oxygen consumption measured during intense exercise, and it represents the maximum integrated capacity of the pulmonary, cardiovascular, and muscle system to uptake, transport, and utilize oxygen [8,22]. VO2max can be measured in absolute liters of oxygen consumed per minute (L/min) or relative to weight in milliliters of oxygen consumer per kilogram of body mass per minute (mL/Kg/min). VO2max is a physiological characteristic bounded by the parametric limits of the Fick Equation, which states the following: [V̇o2 = Q̇ × (a-v)O2 diff], where Q stands for cardiac output, which can be calculated as stroke volume multiplied by heart rate and (a-v)O2 diff represents the arteriovenous oxygen difference [23]. According to the Fick equation, every change in VO2max is matched by a concomitant change in maximal cardiac output or arteriovenous difference [24]. If VO2 reaches a maximum value, termed VO2max, when work done above critical power is performed, and as W’ is depleted, it would indicate that there may be a causal relationship between critical power and oxygen delivery and extraction. If this were the case, then there should be evidence that increasing an individual’s VO2max will come with a concomitant upward shift in critical power.
According to Hill, Long, and Lupton, “In running the oxygen requirement increases continuously as the speed increases, attaining enormous values at the highest speeds; the actual oxygen intake; however, reaches a maximum beyond which no effort can drive it. The oxygen intake may attain its maximum and remain constant merely because it cannot go any higher owing to the limitation of the circulatory and respiratory system” [25]. Since then, there has been additional evidence supporting the belief that the pulmonary system can be a limiting factor in maximal effort exercise. For example, in elite athletes with very high maximal cardiac outputs, the decreased transit time of red blood cells in the pulmonary capillaries can lead to a pulmonary diffusion limitation. This was demonstrated in 1965 when the former mile world record holder Peter Snell performed a maximal treadmill step test, where he finished with a SpO2 level of 80% [26]. Additionally, this finding was later confirmed by Dempsey et al. and Powers et al. when they showed that arterial oxygen desaturation occurs in some highly trained endurance athletes and when these subjects breathe hyperoxic gas mixtures, their hemoglobin saturation and VO2max increase [27,28]. In 2010 Vanhatalo, et al. found that the powerduration curve asymptote was shifted upwards when exercise was performed while breathing hyperoxic air [4]. On the other hand, Dekerle et al. demonstrated that it was shifted downwards when exercise was performed while breathing hypoxic air [29]. These experiments involved the within‐subject manipulation of arterial oxygen content via hypoxic and hyperoxic inspired gas, inferring altered O2 delivery to exercising muscle. They demonstrated that critical power was increased with hyperoxia and decreased with hypoxia. These lend support for the relationship between critical power and oxygen transport given that oxygen transport to the skeletal muscle is a product of both cardiac output and arterial oxygen saturation, both of which can be limiting factors for VO2max [22]. Dekerle, et al. has already shown that reductions in oxygen transport, through breathing hypoxic air, result in a downward shift of the power-duration asymptote [29]. However, in order to further substantiate the relationship between oxygen transport and critical power, there should be evidence that reductions in blood flow will cause a similar downward shift (Figure 2).
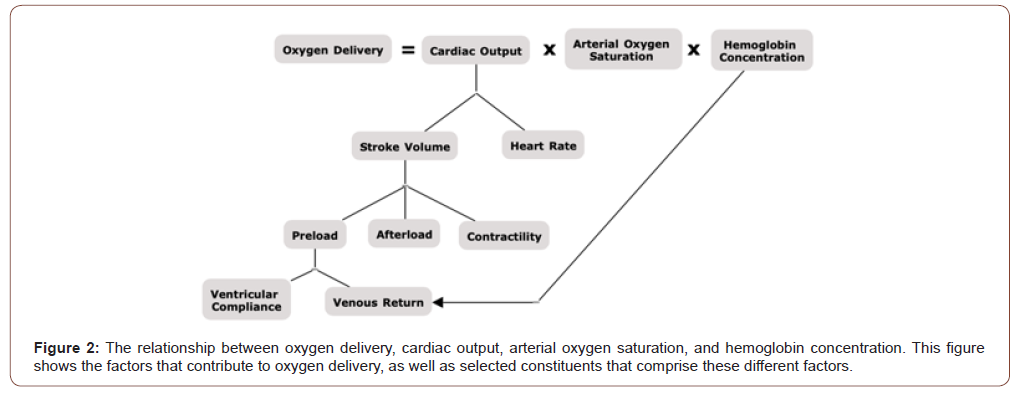
During occlusion exercise, where blood flow is restricted, the Fick equation, [V̇o2 = Q̇ × (a-v)O2 diff], states that VO2max is proportional to oxygen extraction. Given the speculative relationship between oxygen delivery and critical power, Monod & Scherrer postulated that blood flow occlusion during exercise would reduce critical power to zero watts without altering the curvature constant W’ [3]. This was later empirically tested by Broxterman and colleagues who assessed the influence of blood flow occlusion on critical power, W’, and muscle oxygen kinetics. In this case, it was found that the reduction in oxygen delivery with blood flow occlusion decreased critical power to zero watts and led to an increased W’ compared with the control group [30,31]. These findings support the aerobic nature of critical power and demonstrate that the amount of work that can be done above critical power can vary across conditions. Moreover, the amount of work that can be done above critical power appears to be a consequence of the depletion of intramuscular energy stores and the accumulation of fatigue-inducing metabolites, limiting exercise tolerance and determining W’ [30,31]. Based on these findings, it appears that reductions in blood flow, and subsequently, oxygen delivery, lower critical power, resulting in the utilization of W’ and fatigue at lower relative intensities. Additionally, the larger body of evidence suggests that any alteration in oxygen delivery, whether through blood flow changes or inspired oxygen concentration changes, will directly affect critical power and W’ depletion [4,29,32,33].
The impact of oxygen delivery on the curvature constant, W’, and it’s reconstitution
The curvilinear relationship between power output and the time for which it can be sustained is a fundamental and well-known feature of high-intensity exercise performance. This relationship ‘levels off’ at a ‘critical power’ that separates power outputs that can be sustained with stable values of, for example, muscle phosphocreatine, blood lactate, and pulmonary oxygen uptake, from power outputs where these variables change continuously with time until their respective minimum and maximum values are reached and exercise intolerance occurs. The amount of work that can be done during exercise above critical power is known as the W’. The W’ is constant but may be utilized at different rates depending on the exercise power output’s proximity to critical power. As a result, the W’ represents a fixed amount of work that can be performed above critical power before exhaustion ensues [34]. The mechanistic bases of the W’ are complex and remain ambiguous. The W’ was originally described as an anaerobic work capacity [3]. Recent evidence has shown that W’ is sensitive to manipulations in oxygen delivery and extraction via blood flow occlusion and alterations in the muscle contraction duty cycle [4,30,31]. Additionally, it appears that the amount of work that can be performed above critical power does not appear to be a determinant of W’, but rather a consequence of the depletion of intramuscular energy stores like phosphocreatine and glycogen, and oxygen, as well as the accumulation of fatigueinducing metabolites like Pi and H+, which limit exercise tolerance and determine the W’ [9,19,35,36].
W’ is expended when power output exceeds critical power and it is reconstituted when the power output is below critical power [34,37]. However, if W’s reconstitution during relaxation phases between bouts of work done above critical is insufficient, a net depletion of W’ will occur and when W’ is fully depleted task failure ensues [38]. This depletion of W’ may be causally linked to insufficient re-oxygenation of the muscle during the periods of relaxation. While W’ will be used in its entirety for exercise intensities above critical power, the proportion of W’ that contributes directly to external work is not constant across all power outputs. For example, at rest under occlusion W’ will be used it its entirety to support factors distinct from external work like resting cellular processes and ion handling, which is demonstrated by resting blood flow occlusion leading to myoglobin desaturation, increases in adenosine diphosphate, and decreased phosphocreatine [39,40]. As power output increases, a greater proportion of W’ would be utilized for external work, though it appears that some of the energy derived from the utilization of W’ still contributes to factors that are distinct from external work [30,31].
In an investigation by Broxterman et al., it was found that W’ was reduced following resting blood flow occlusion, which would impede oxygen delivery [30,31]. Studies have also demonstrated that hemoglobin and myoglobin deoxygenation occur during resting occlusion as well, which subsequently phosphocreatine as well [39,41]. Based on the aforementioned evidence, we can infer that W’ is tightly correlated with oxygen delivery and availability such that a very low muscle oxygenation saturation (SmO2) is an indication of depleted W’. If that were the case, then SmO2 should be a good marker to predict task failure. Based on Feldman and colleagues’ findings, who found that maximum and minimum values of deoxyhemoglobin and oxyhemoglobin respectively were correlated with a loss of force production, this appears to be the case [42].
Oxygen Delivery as a Rate Limiting Factor for Critical Power and W’
VO2max as a measure of integrated capacity
VO2max refers to the maximum rate of oxygen consumption measured during intense exercise. VO2max can be measured in absolute liters of oxygen consumed per minute (L/min) or relative to weight in milliliters of oxygen consumer per kilogram of body mass per minute (mL/Kg/min). The concept that there exists a finite rate of oxygen transport from the environment to the mitochondria of exercising muscles began with Archibald Hill and Hartley Lupton [42]. Since then, VO2max has become one of the most ubiquitous measurements in all of exercise science. VO2max is a physiological characteristic bounded by the parametric limits of the Fick Equation, which states the following: VO2max = Q*[CavO2], where Q stands for cardiac output, which can be calculated as stroke volume multiplied by heart rate and Ca-vO2 represents the arteriovenous oxygen difference [23].
The best-accepted method for measuring VO2max is the cycle ergometer ramp test completed to exhaustion, though other modalities can be used effectively. This test involves exercising at an intensity that increases every few minutes until the participant reaches volitional failure at a maximal exertion point. During this test, a participant will wear a face mask to measure the volume of gas concentrations of inspired and expired air. It’s important to note that an individual’s maximum attainable oxygen consumption rate will vary slightly based on the specific protocol they are tested with and the modality they are tested on. As a result, it’s important to conceptualize VO2max as a range of values rather than a single discrete number for each individual.
Classical views of VO2 max emphasize its critical dependence on convective oxygen transport to the working muscles. However, we cannot neglect the fact that oxygen delivery is a product of both blood flow and arterial oxygen saturation. Additionally, VO2max can be limited by multiple different physiological variables, including pulmonary diffusion capacity for oxygen, maximal cardiac output, peripheral circulation, and skeletal muscle’s metabolic capacity. As a result, VO2max can be conceptualized as the pulmonary, cardiovascular, and muscular systems’ maximum integrated capacity to uptake, transport, and utilize oxygen, respectively [8].
The impact of hypoxia and heavy exercise on oxygen delivery, VO2max, critical power, and W’
During exercise, the circulatory system is challenged to improve oxygen delivery to the working tissues. Both convective and diffusive factors regulate oxygen delivery. Convective oxygen transport refers to the bulk movement of oxygen in the blood and depends on active, energy-consuming processes that generate flow in the tracheobronchial tree and circulation [44]. Diffusive oxygen transport refers to the passive movement of oxygen down its concentration gradient across tissue barriers, including the alveolar-capillary membrane and the extracellular matrix between the tissue capillaries and individual cells mitochondria [44]. During exercise in hypoxia and at exhaustion, the circulatory system is challenged to facilitate oxygen delivery to the working tissues, which ultimately impacts the development of fatigue and performance.
As altitude increases, there is an expected and logical decrease in convective oxygen transport [45]. Specifically, there is a decreased peak oxygen uptake in the pulmonary system and systemic circulation and a reduction in pulse oxygen saturation at the capillaries level. At and above 3,800m, maximal heart rate is also decreased [46]. Given that VO2max is determined through a combination of central factors, like stroke volume and heart rate, as well as peripheral factors like arterial oxygen saturation, it is logical that VO2max is meaningfully decreased at altitude and in hypoxia, which has been previously observed [47-49]. These convective oxygen delivery changes can also occur through modifications in blood flow, and they can even be induced in a healthy human exercising heavily at sea level [45]. As exercise increases up to a heavy exertion level or close to a maximal power output, there is a progressive decrease in muscle oxygen saturation to a minimal point or plateau, which gives rise to exhaustion [50,51]. In hypoxic conditions, this plateau or bottoming out in oxygenated hemoglobin concentration is considered an indication of maximal skeletal muscle oxygen extraction as a product of reduced oxygen availability [52]. These oxygenation and hemodynamics changes can be observed non-invasively with near-infrared spectroscopy in live time [53].
Consistent with the data on hypoxia’s dose-response effect on VO2max, there is a curvilinear decrease in critical power under hypoxia conditions [54]. It is important to note that VO2 at critical power is below VO2max, and that critical is associated with the highest exercise intensity where a VO2 steady state occurs [18,19,55]. The VO2 steady state indicated the highest intensity where a ‘metabolic stability’ can be achieved, where metabolic stability is characterized by minimal disturbances to intramuscular phosphocreatine stores among other factors [50]. There is evidence supporting the idea that phosphocreatine is not only reconstituted via oxidative means but dependent on oxygen availability. In hypoxia, convective oxygen transport to the working muscles occurs, and the VO2 primary component decelerates [56]. Since the VO2 primary component is considered an ‘epiphenomenon’ of metabolic stability and has been shown to correlate with critical power, then an oxygen supply and delivery limitation on VO2 kinetics may impair metabolic stability and thus explain why critical power is reduced in hypoxia [29,57,58].
Since critical power is lower under conditions of hypoxia, then it holds that a given absolute intensity will cause a faster depletion of W’ under these conditions. According to the critical power model, faster depletion of W’ will cause a reduction in time to exhaustion at a given fixed power output above critical power. This would be associated with an exacerbated rate of fatigue development in hypoxia, which has been demonstrated by Romer, et al. [59]. Hence, rather than conceptualizing hypoxia per se as the mechanism which exacerbates fatigue, it is the effect of hypoxia on decreasing critical power that leads to a more rapid onset of fatigue coinciding with the depletion of W’ at a given fixed absolute power output. However, there is also evidence that W’ will be reduced at altitude and that changes in W’ are related to changes in critical power relative to VO2max, which suggests that W’ isn’t simply an aerobic energy store as was once believed [58]. Additionally, the effect of hypoxia on W’ appears to display a threshold characteristic since there is no significant change in W’ at a lower altitude, but past 4,250m meaningful reductions were observed [58]. Valli, et al. has suggested that the decrease in W’ at altitude is consistent with reduced muscle-venous oxygen storage [60]. This seems plausible given the decreased peak oxygen uptake that occurs at altitude, which results in lower arterial oxygen saturation and as well as lower muscle oxygen saturation. Both of these have been shown to cause a direct decrease in VO2max and critical power. This suggests that oxygen uptake and transport can be rate-limiting factors that determine critical power and W’.
Modeling Time to Exhaustion
Using muscle oxygen saturation as estimation of the power duration relationship
The critical power concept describes the relationship between sustainable power output and severe intensity exercise duration above the ‘critical power’. A simple hyperbolic, two-parameter model was proposed to explain this concept: t lim=(W’)/(P-CP) where t lim is the time until task failure occurs, P is power output, CP is the critical power defined as a rate-limited sustainable power output below which no expenditure of W’ occurs, and W’ is the total work accumulate above critical power until task failure. In this model, the value W’ is progressively depleted during exercise whenever power output exceeds critical power and reconstitutes when power output falls below critical power [61]. Additionally, if W’ is depleted to zero, then t lim also reaches zero, and hence task failure ensues. Since the total amount of work accumulated above critical power is terminated at the moment of task failure, the mechanisms leading to task failure also determine W’s value. It has previously been observed that as exercise increases up to a heavy exertion level, or close to a maximal power output, there is a progressive decrease in muscle oxygen saturate to a minimal point or plateau which gives rise to exhaustion [50,51]. Given this information, and the relationship between oxygen delivery, critical power, and W’ it stands to reason that muscle oxygen saturation can be used as an indicator of proximity to failure. Previous investigations have shown that isometric muscle contractions result in the same SmO2 value at the point of failure for individuals [42]. These results can be further extrapolated to confirm the relationship between time to task failure and time to SmO2 minimum [42]. Considering these findings a value as simple as SmO2min and SmO2 rate of change can be used to potentially predict failure during isometric muscle contraction and similar results were reported during sustained gripping tasks where maximum and minimum values for deoxyhemoglobin and oxyhemoglobin respectively were correlated with loss of force production [42]. While more research into this area is needed before this concept can be extrapolated to cyclic activities such as running, it seems promising given that the hyperbolic form of the power duration relationship is rigorous and highly conserved across forms of exercise, individual muscles, and muscle groups [10]. Additionally, experimental evidence suggests that when oxygen delivery is fixed via blood flow occlusion, regional oxygen saturation predicts time to exhaustion [62]. This aligns with the idea that tissue oximeters provide information on the balance between oxygen supply and oxygen demand and that regional oxygenated hemoglobin saturation represents tissue reserve capacity following tissue oxygen extraction [17]. As a result, the equation t lim= W’/P-CP can be reconceptualized as t lim= (regional O2Hb saturation)/(the balance of oxygen supply and demand) [16,62]. However, regional oxygenated hemoglobin saturation fails to predict time to fatigue accurately when delivery is not fixed via blood flow occlusion. As a result, more research needs to be done in order to further elucidate the incompletely understood relationship between oxygen delivery, critical power, and W’.
Conclusion
These findings and many others, strongly suggest a causal relationship between oxygen delivery and critical power, such that muscle oxygen saturation may be used to approximate the powerduration relationship non-invasively and in real-time. While critical power can be accurately used to predict time to exhaustion at a fixed power-output above CP, one of its major limitations is its sensitivity to environmental factors like altitude and prior heavy exercise, fatigue, and glycogen depletion. As a result, this requires an athlete to test critical power in all of these conditions, which may not be feasible. By using minimum muscle oxygen saturation values and rate of change of muscle oxygen saturation, it’s possible that these issues are voided. However, further research is needed to examine the relationship between critical power and oxygen delivery, W’ and regional oxygenated hemoglobin saturation, as well as time to exhaustion, minimum muscle oxygen saturation, and rate of change of muscle oxygen saturation.
To read more about this article...Open access Journal of Anatomy & Physiology
Please follow the URL to access more information about this article
To know more about our Journals...Iris Publishers
To know about Open Access Publishers
No comments:
Post a Comment